Current interests
Cosmology deals with the question of what our Universe as a whole is made of, as well as its history and evolution. The answers and models that this field provides set the stage for the formation of galaxies, stars, and planets. Two of the most pressing mysteries in cosmology are the nature of
dark matter and
dark energy, which appear to make up
96% of the Universe's current energy budget. Another big question is the
origin of structure in the universe, the most popular hypothesis being an early epoch of rapid accelerated expansion,
inflation. On the other hand, we are faced with the challenge of attempting to extract the best possible constraints on dark matter, dark energy, and inflation from
complex and expontentially growing observational data.
One of the fascinating aspects of cosmology is the rich variety of observations that can be used to probe dark matter, dark energy, and gravity. I am currently focused on galaxy clustering, stellar streams in the Milky Way, and massive galaxy clusters, but in the past also spent substantial efforts in gravitational lensing.
New inference methods
Galaxy surveys allow us to map out a large part of the observable universe, by supplying us with positions of millions of galaxies (see figure above). How do we use these to extract insights on dark matter and dark energy? We know that galaxies broadly trace the distribution of matter (including dark matter), but the detailed relation is extremely complicated and also depends on the type of galaxy and their formation history. Our group has developed an
effective theory of the galaxy-matter relation, and implemented this in a fast differentiable code (
LEFTfield) which generates simulated galaxy maps, given parameters that describe dark matter and dark energy, and the statistics of the initial conditions.
This code offers several new pathways for learning about dark matter, dark energy, and inflation. The direct approach, field-level inference, attempts to infer the joint posterior of cosmological parameters and the entire initial seed fluctuations. This is a very challenging, million-dimensional inference problem which we tackle with Hamiltonian Monte Carlo sampling (and generalizations). While hard, this consistent Bayesian approach is guaranteed to yield optimal constraints in the context of the model considered. The figure from this paper illustrates results from one such inference chain. The left panel shows the (simulated) data used as input, while the middle shows the mean of the model prediction, showing good agreement; the residual (right panel) is consistent with noise.
An alternative, less expensive approach is to first compress the galaxy map into summary statistics that we want to be as sensitive as possible to cosmological physics. The classical approach involves 2- and 3-point correlation functions, or power spectrum and bispectrum. LEFTfield however allows us to couple our robust effective model for galaxies to a much wider range of summary statistics, by making use of simulation-based inference (SBI). In this approach, we train a deep neural network (autoregressive normalizing flow) to learn the joint posterior of model parameters and summary statistics, which is then evaluated on the summaries of the observed data. We are currently working on devising more powerful summaries than used in the field so far.
Machine Learning
Technologies from artificial intelligence/machine learning are likely to have a transformative impact on astrophysics, given that it is a data-driven field. We are already using these when doing simulation-based inference (see above); in this case, we are straightforwardly using a deep neural network as flexible (and invertible) function approximator in a supervised learning setting. The range of AI/ML applications in the field will certainly be much broader in the future, including attempts to build foundation models and performing AI-assisted discovery. In this regime, being able to
interpret the result of AI models will be crucial. This is the aspect of machine learning I am personally most interested in, and have started to
work on recently.
Dark matter
By definition, dark matter is (essentially) dark, so the only guaranteed way to probe it is via its gravitational effects. Many dark matter scenarios make the same prediction for the large-scale distribution of dark matter, such that the cosmological constraints inferred from the galaxy maps mentioned above are largely independent of what dark matter is. However, different kinds of dark matter behave very differently on small scales, that is within gravitationally bound structures (galaxies and galaxy clusters). Making robust predictions in this regime is much harder, and astrophysicists try to identify relatively clean systems that can be modeled reliably.
One example are
stellar streams in the Milky Way which arise when globular clusters are slowly tidally stripped.
Thanks to their very small velocity dispersion, they can be used as a sensitive
gravitational probe of structure in both dark and visible matter, and hence to possibly distinguish between different dark matter scenarios.
We have developed a
detailed semianalytical understanding for how (possibly dark) structures in the Milky Way leave imprints in stellar stream, identifying three stages of evolution, which can guide astrophysicists to look for the most promising streams to use as probe of dark matter.
Dark Energy vs Modified Gravity
Observations through Supernovae and other probes have shown that
the expansion of the Universe is accelerating. This is a big puzzle for cosmologists, since according to our current understanding of gravity (through
Einstein's theory of General Relativity), a Universe filled with just matter and radiation (light) should be
decelerating.
Different explanations of this mystery have been postulated: it could be due to a cosmological constant, the simplest explanation which is still consistent with current observations. It could be due to an exotic form of (loosely speaking) anti-gravitating energy, dubbed Dark Energy, which effectively drives the Universe apart. Or, finally, General Relativity could not be the final word on gravity.
One of the goals of my research has been to explore how a Universe governed by a modified form of gravity would look like, and how we can use observations to test the behavior of gravity over huge distances. One important tool are simulations of structure formation, where a large, representative chunk of the Universe is followed through cosmic time, starting from the tiny initial density fluctuations that we see imprinted in the cosmic microwace background. The picture above shows a slice through such a simulation which was run to the present time. The black dots show collapsed structures of dark matter, which host groups and clusters of galaxies (though our simulations do not have sufficient resolution to actually see galaxies form).
The field of dark energy science could also be more interesting than widely assumed so far, since typically dark energy is supposed to be perfectly homogeneous. Recently, I have become involved in efforts studying the evolution of clustering dark energy, a theoretically well-motivated scenario where dark energy develops significant inhomogeneities as the growth of structure in the Universe proceeds.
Galaxy Clusters
Galaxy clusters are the
most massive, gravitationally bound structures in the Universe. Massive clusters, which can contain thousands of galaxies, are exceedingly rare, so clever methods are needed to find them in large sky surveys. Apart from identifying them
as ensembles of galaxies with optical telescopes, they can also be searched for in X-rays: the tenuous diffuse gas in massive clusters is heated to millions of degrees by the enormous gravitational pull, making it shine in high energy X-rays. Clusters can then be found and studied in detail by X-ray satellites such as
Chandra and
XMM-Newton. The picture here shows the cluster Abell 3376 as seen in X-rays (yellow), radio (blue), and the optical band (white). Please click on the picture to read more.
The scarceness of massive clusters is also a virtue: their abundance responds sensitively to changes in the growth of structure. Modifications to the behavior of gravity typically have a large impact on cluster abundance. In 2009, we used a sample of X-ray emitting clusters together with our simulation results to constrain a modified gravity scenario called f(R) gravity (which attempts to explain the acceleration of the Universe without dark energy). This work, which was featured in a Chandra press release, is the result of a collaboration with Wayne Hu from the University of Chicago and Alexey Vikhlinin from the Harvard/Smithsonian Center for Astrophysics. Please read on at the Chandra site !
The above results are of course from a while ago. We are working on updating modified gravity constraints from the much larger recent galaxy cluster samples obtained by using the Sunyaev-Zel'dovich effect on the cosmic microwave background. To be updated soon!
Unraveling the initial conditions of the Universe
Many observations, in particular the cosmic microwave background, have now demonstrated beyond a doubt that the Universe started out from a very hot dense and homogeneous state (the “Big Bang”). But why was the Universe so homogeneous (it still is on very large scales today), and how were the initial fluctuations generated that grew into the clusters, galaxies, stars and planets today ? The leading theoretical contender is the scenario of
inflation: the Universe underwent a period of very rapid expansion early on in its history (corresponding to extremely high energy scales).
Quantum fluctuations were stretched out and “froze in”, later to become the seed fluctuations out of which all structure formed. Of course, we would like to test this scenario and obtain clues on if, how, and when inflation happened. One possibility is to look for hints in the statistical distribution of the initial fluctuations, in particular for correlations between fluctuations on different length scales. Technically, this is called
primordial non-Gaussianity. One of the most fascinating aspects of cosmology today is that, through primordial non-Gaussianity, we can
study inflation using the distribution of galaxies on large scales, such as observed by the Sloan Digital Sky Survey (above). In another words, we can use the largest observable scales in the Universe, to probe physics at energy scales orders of magnitude beyond particle accelerators on Earth !
In order to do this however, we need theoretical models that connect the statistics of the initial conditions predicted by inflationary models with the galaxy distribution today. This is an area of ongoing, intense research, which we base on the effective theory of galaxy formation mentioned above.
Gravitational waves and general-relativistic effects
The large-scale distribution of galaxies, and their shapes, also offer opportunities to search for
gravitational waves from inflation, the echo of the Big Bang, which we
pointed out for the first time. To do this however, we need to embed our physical model for structure and galaxy formation into the context of Einstein's theory of General Relativity, since gravitational waves are a purely relativistic effect. I have a long-standing interest in this field. We recently
laid out a path describing how we can systematically embed the physical model of galaxy formation into General Relativity, by mapping from the coordinates that a local observer in the respective galaxy would choose, to those that a distant observer (on Earth) uses (see sketch).
Past research
Gravitational lensing
(2012) Gravity affects not only ordinary matter, but light as well. The intense gravitational pull of a massive foreground galaxy or cluster can distort the image of galaxies that lie far behind the "lens". The neat property of this
gravitational lensing is that it responds equally to all matter. Thus, even the otherwise elusive
dark matter shows up in this effect, making it especially interesting for cosmologists. The picture on the right shows a very distant galaxy image (blue) strongly distorted by a galaxy in the foreground (yellowish). Click on the image to obtain more information.
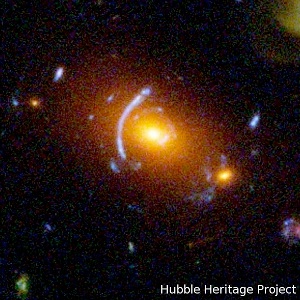
One particular technique, called weak lensing aims to measure the large-scale structure of the Universe through the distortions of background galaxies. Weak lensing is another promising avenue for probing gravity in cosmology, and has been hailed as potentially the most powerful observational tool in cosmology. The main downside is the smallness of the effect, distorting any individual background galaxy image by a few percent or less, requiring great care when attempting to measure the effect. One of the subtleties is that even the way these background galaxies are selected has an impact on the measurement. This effect which I discovered in 2009 together with colleagues from Chicago, Columbia University, and Brookhaven National Lab, has to be taken into account for upcoming ambitious surveys, such as the Dark Energy Survey. Please see this "News and Views" article in Nature for a discussion and background.
We have also recently detected weak lensing magnification in the COSMOS survey, using images taken by the Hubble Space Telescope. (...)
Cosmic rays
(2009) I have previously worked on ultra high energy cosmic rays, and was a member of the Pierre Auger collaboration. In particular, I have worked on figuring out the composition of the cosmic rays (are they protons, or heavier nuclei ?) and on comparing hadronic interaction models with the data.
Here are some pictures of simulations of cosmic ray-induced air showers, for various primary particles and energies.